July 1st, 2011
Key Findings
- Aerosol-cloud interactions have been included in a GFDL climate model for the first time. Aerosol activation is physically based, depending on aerosol composition, size distribution, and updraft speed. Updraft speeds are represented by sub-grid distributions of vertical velocity for stratiform and shallow cumulus clouds.
- Aerosol optical depths and scattering properties (and clear-sky downward surface shortwave fluxes) are simulated more realistically in AM3 than in GFDL’s earlier climate model, CM2.1. Despite the implied improvements in direct forcing in AM3, its warming from pre-industrial to present-day conditions is somewhat less than observed.
- AM3’s present-day climate compares favorably with models in phase 3 of the Climate Model Intercomparison Project. It’s shortwave cloud forcing, Arctic sea-level pressure, and Amazon precipitation are improved over AM2, and CM3 has more realistic Arctic sea ice than CM2.1
- CM3 captures major trends in stratospheric ozone, including the Antarctic ozone hole, during the late 20th century.
Earlier generations of climate models at GFDL have specified cloud properties to be independent of atmospheric aerosol composition, despite fairly well-understood (at least for liquid clouds) dependence of cloud droplet number on aerosol composition and size distribution. Cloud drop number in turn exerts a major control on cloud radiation, notably albedo, and microphysics and macrophysics, notably cloud structure and lifetimes. Aerosol-cloud interactions are likely to be important in anthropogenic climate change. They have been difficult to incorporate in climate models because they occur at scales much smaller than resolved by climate models. CM3 parameterizes these smaller scales using probability distribution functions for motions smaller than those it explicitly resolves.
Aerosol-cloud interactions due to anthropogenic aerosol changes cool the Earth. The last two decades of the twentieth century warm by 0.32 C relative to 1881-1920 in CM3. CM2, which did not include cloud-aerosol interactions, warms by 0.66 C. Observational warming estimates range from 0.52 to 0.56 C. Figures 3 and 4 from this paper show that aerosol concentrations and absorption properties are simulated more realistically in CM3 than CM2. The direct effects of these aerosols are thereby more realistically simulated in CM3 than CM2. Figure 5 from this paper shows the clear-sky downward surface shortwave fluxes. These fluxes are biased low in CM2, indicating excessive aerosol direct forcing, consistent with the high bias in CM2 optical depths relative to observations. Note that even with cooling aerosol direct effects too large compared to observations, CM2 warmed too much over the twentieth century. On the other hand, CM3, whose aerosol direct effects are more realistic relative to observations but also includes cloud-aerosol interactions, does not warm enough over the twentieth century.
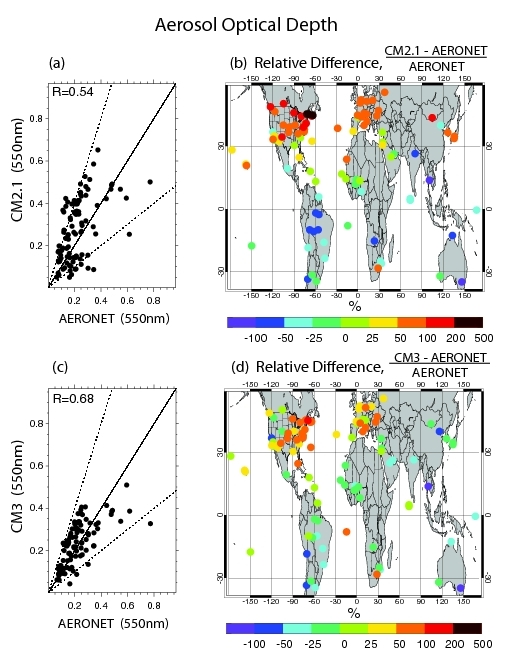
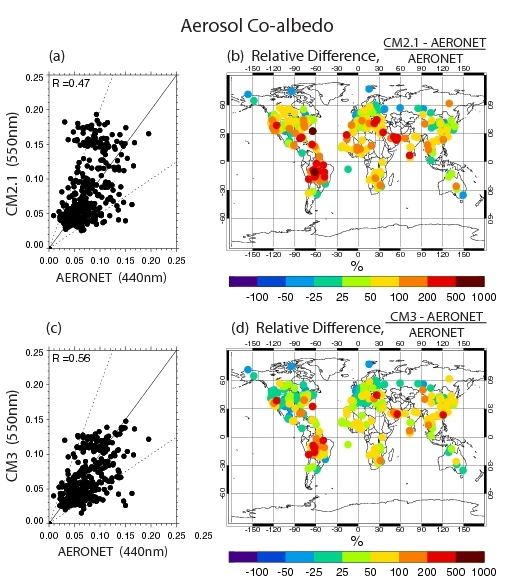
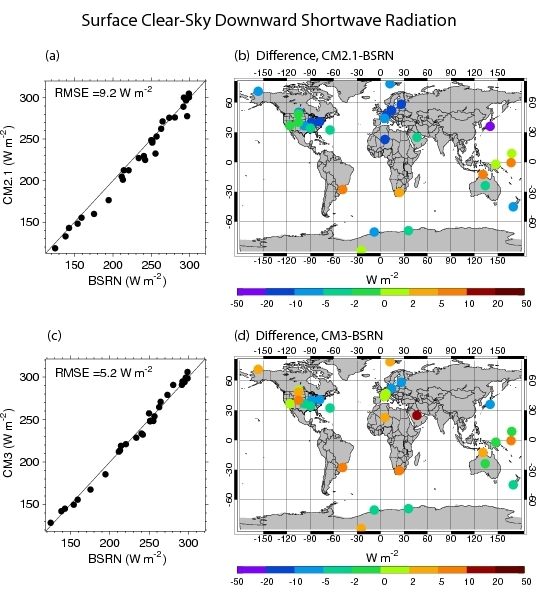
For more information: Donner, Leo J., Bruce Wyman, Richard S Hemler, Larry Horowitz, Yi Ming, Ming Zhao, J-C Golaz, Paul Ginoux, Shian-Jiann Lin, M Daniel Schwarzkopf, John Austin, G Alaka, W F Cooke, Thomas L Delworth, Stuart Freidenreich, C Tony Gordon, Stephen Griffies, Isaac Held, William J Hurlin,Stephen A Klein, Thomas R Knutson, A R Langenhorst, H C Lee, Y Lin, B I Magi, Sergey Malyshev, V Naik, Mary Jo Nath, R Pincus, Jeff J Ploshay, V Ramaswamy, Charles J Seman, Elena Shevliakova, Joseph J Sirutis, William F Stern, Ronald J Stouffer, R John Wilson, Michael Winton, Andrew T Wittenberg, and Fanrong Zeng (2011), The dynamical core, physical parameterizations, and basic simulation characteristics of the atmospheric component AM3 of the GFDL Global Coupled Model CM3. Journal of Climate, 24(13), doi:10.1175/2011JCLI3955.1.